Researchers develop new and direct method to study singularities
Incoming Yale assistant professor and fellow researchers at University of California, Berkeley use an ultra-cold atom approach to study singularities in the band structure of materials.
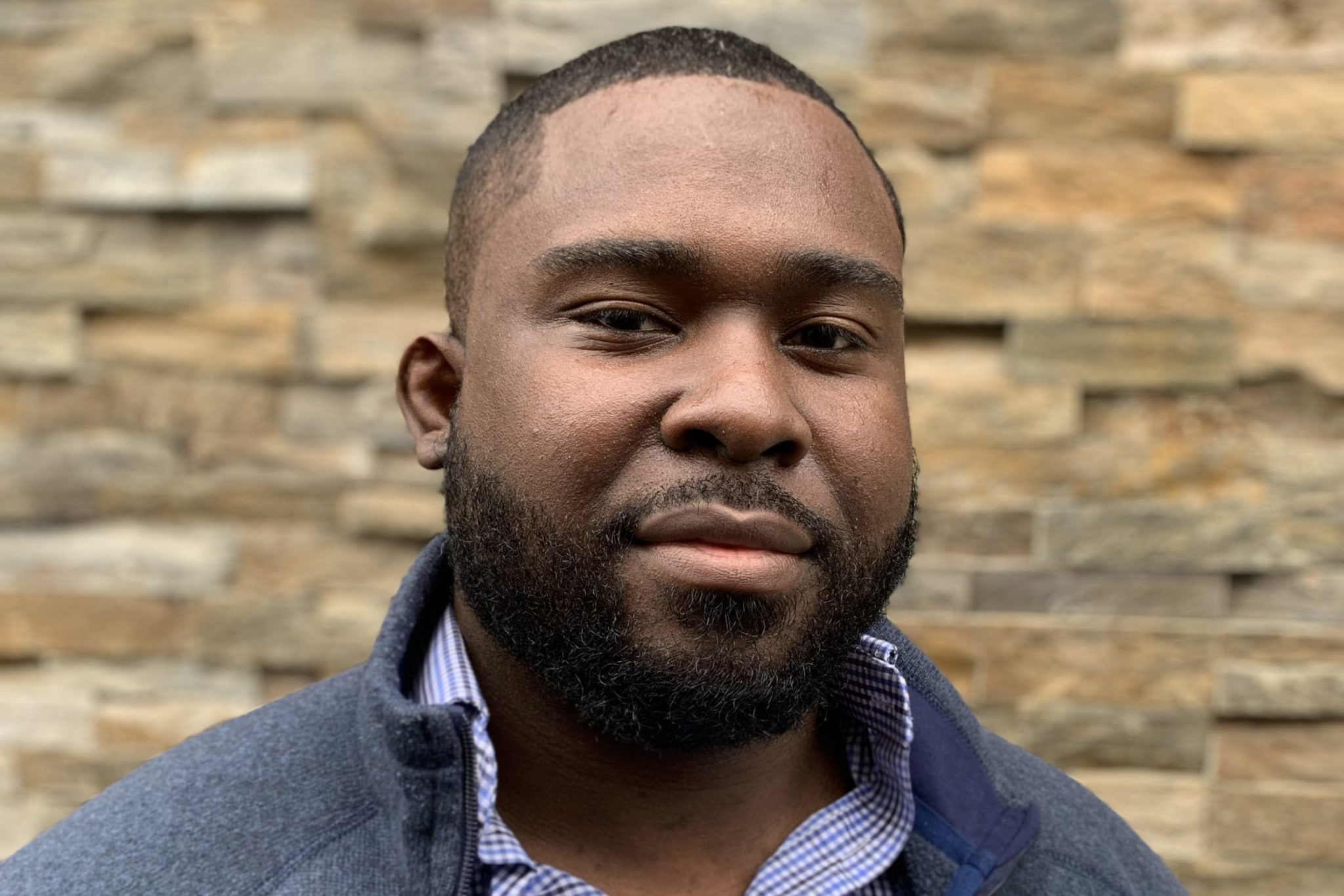
Courtesy of Lydnsey McMilon-Brown
An incoming assistant professor of physics has discovered a new experimental setup that could make understanding physical materials easier.
Researchers from the University of California, Berkeley, including Charles Brown II, an assistant professor of physics planning to join Yale faculty in January 2023, found a new and more direct method to examine singularities in the band structure of materials. This method has the potential to enhance our understanding of the properties of the materials themselves.
The research, published in Science, employed quantum simulation to derive the results in this quantum simulation. They used lasers to imitate a crystal lattice and ultra-cold atoms of rubidium-87 to imitate the electrons in the lattice. Then, they accelerated the rubidium atoms into a singularity in the energy band structure — where two energy bands meet — and turned them at an angle before accelerating them away. This changed the proportion of atoms in different energy bands depending on the turning angle.
“We’re using a simpler system over which we have a lot of control to emulate condensed matter systems, like real physical crystals, for example, graphene,” Brown said. “Using systems like the ones I work on, where you make an artificial crystal out of light … by studying how atoms hop around that artificial crystal, you can, in many ways, exactly emulate how electrons hop around in solid piece of graphene, and so you can also emulate the interesting physical properties of this material.”
From the resulting proportion, researchers can work out some interesting properties of the band structure of the material, which can later be related to topics like superconductivity and quantum magnetism.
Vladyslav Kozii, a member of the study’s theory team and assistant professor at Carnegie Mellon University, clarified that this emulation is possible because the equations of motion that govern the atoms in this optical lattice are the same as the ones that govern electrons in a crystal lattice.
This experimental set-up was used to study the singularities in the band structure of the lattice. Band structure depicts the ranges of energies that particles can and cannot occupy in a lattice, and singularities are points where two energy bands touch — where they are at the same energy level.
“The concept we were playing with is that you figure out the geometry of the space you’re in, … whether there’s hills or valleys, by walking through the space,” said Dan Stamper-Kurn, the principal investigator of the experimental group, “That was the idea of this experiment — that by transporting the atoms through the lattice, we can figure out the geometry of the space it was transported through.”
In this study, the team accelerated the rubidium atoms into such singularities in the optical lattice. Aleksandr Avdoshkin, a graduate student in the theory team, explained that instead of moving the atoms, it was easier to move the optical lattice itself so that the atoms moved relative to the lattice.
Once the atom was in the singularity, the lattice was turned. The atoms were accelerated away, but now the proportion of atoms in each energy band had changed. At the singularity the bands have the same energy, and when moving through that point, some atoms transferred from lower to higher energy, for example. Then, the proportion of atoms in each band was measured. The researchers observed that the proportion depended solely on the turning angle, and making one full rotation resulted in the proportion making oscillations as well, with the number of oscillations per rotation depending on the type of singularity the atoms were passing through.
Shao-Wen Chang, a graduate student in the experimental team, described that there are two types of singularities, linear and quadratic, differentiated by the rate at which the energy difference between the two bands grows with distance from the singularity.
At a linear singularity, the proportion of atoms in each band underwent one full oscillation for each 360 degree rotation of the angle, while at a quadratic singularity, it underwent two full oscillations for each rotation, as seen in the part C’s of the diagrams below. This information can be used by scientists to determine some important properties of the material.
According to Brown, the linear band-touching points (singularities), called Dirac points, had been studied extensively prior to this experiment. However, the quadratic ones had not been, which was partly because it was difficult to probe them using earlier methods. Thus, one important aspect of this study is that scientists could examine those points directly.
“There are all these exotic physics effects theorized to be associated with quadratic band-touching points, different topological effects, maybe even superconductivity and exotic quantum magnetism,” said Brown. “But, in my field, there hadn’t been a good technique to study them until now. But we knew we needed to study them because quadratic band-touching points appear in raw materials so understanding them better helps us understand how many interacting particles subject to quantum mechanics work.”
The cold-atom quantum simulation approach allows a way to probe the singularities simply and directly by changing the setup of the lasers, which Kozii said, isn’t something you can do with actual solid-state systems at all.
The researchers all stressed that their work developed a new tool that can help understand singularities in band structure, which give rise to a lot of the materials’ properties and can be important to atomic physics and material science.
“You can do manipulations with physical systems which could not be imagined a few years ago — I think that’s really cool,” Kozii said.
This study was published in Volume 377, Issue 6612 of Science.